Translate this page into:
Oxygen sensing
*Corresponding author: Nanduri R. Prabhakar, Institute of Integrative Physiology, Center for Systems Biology of O2 Sensing Biological Sciences Division, University of Chicago, Chicago, Illinois, United States. nanduri@uchicago.edu
-
Received: ,
Accepted: ,
How to cite this article: Prabhakar NR. Oxygen sensing. Indian J Physiol Pharmacol 2022;66:221-5.
Molecular O2 is an essential substrate for energy production. Hence, an adequate O2 supply is critical for mammalian cell survival. Many physiological and pathophysiological situations lead to hypoxia or reduced O2 supply to tissues. Maintenance of homeostasis under hypoxia depends on ‘sensing’ hypoxia and failure to sense O2 can cause severe organ damage and even death. O2 sensing can be ‘innate’ or ‘adaptive.’ While physiological responses to ‘innate O2 sensing’ occur rapidly within few seconds to minutes, ‘adaptive’ O2 sensing is slow in onset often taking a few hours. This editorial provides brief history of discovery, current theories and translational perspectives of ‘innate’ and ‘acquired’ O2 sensing.
INNATE O2 SENSING
As early as 1868, Pflüger, a German physiologist noted hypoxia leads to hyperventilation.[1] Pflüger’s finding spurred interest in identifying structures responding and translating systemic hypoxia to stimulation of breathing. In 1920’s Fernando de Castro at the Cajal Institute, Spain and Corneille Heymans in Belgium independently discovered carotid bodies (CB) as sensory receptors for monitoring arterial blood O2 levels. CB chemoreflex evokes stimulation of breathing by hypoxemia.[2-9] 1938 Nobel Prize in Physiology and Medicine was awarded to Cornielle Heymans. However, the Nobel Citation duly acknowledged the seminal contributions of De Castro in discovering the sensory nature of the CB.[10]
Unlike other mammalian tissues, even a modest decrease in arterial PO2 (from ∼100–80 mmHg) is sufficient for activating CB and the response is rapid occurring within seconds after the onset of hypoxia.[11] Moreover, the increased CB activity is non-adapting and maintained during the entire period of hypoxia.[12] The remarkable sensitivity, the speed with which it responds, with little or no adaptation constitute important characteristics of ‘innate’ O2 sensing by the CB. Considerable evidence suggests that glomus cells are the primary O2-sensing cells of the CB.[11]
Current theories of ‘innate’ hypoxic sensing – Because the response is rapid, innate O2 sensing by the CB utilises existing rather than de novo synthesis of proteins. In 1960s, Lloyd et al.[13] reported brief (1–2 breaths) inhalation of carbon monoxide (CO) inhibits hypoxia-evoked hyperventilation in humans. Because CO binds to haemoglobin more effectively than O2, it was proposed a heme protein(s) might be involved in ‘innate O2 sensing’ by the CB.[13]
Remarkably, CO, once used as a tool to understand O2 sensing by the CB, is now shown to be produced in glomus cells by heme-containing enzyme heme oxygenase (HO)-2.[14] HO-2 is an O2-sensitive enzyme and hypoxia progressively decreases CO production in the CB.[15] HO-2 binds to O2 with low affinity with an apparent Km of 65 ± 5 mmHg (about 80 µM), thereby enabling HO-2 to transduce changes in O2 to changes in CO production. CO inhibits CB sensory nerve excitation by hypoxia.[14] These findings led to the suggestion that low sensory nerve activity during normoxia is due to high CO levels inhibiting CB sensory nerve activity and that hypoxia, by reducing CO production, relieves the inhibition and thereby increase the CB sensory nerve activity.[14]
Recent studies examined how CO inhibits CB sensory nerve activity under normoxia.[15-17] Glomus cells express cystathionine gamma-lyase (CSE), which produces hydrogen sulphide (H2S).[17] H2S, like hypoxia, stimulate the CB sensory nerve activity in rats, mice, rabbits and cats.[18] During normoxia, H2S levels are low and hypoxia increases H2S levels in a stimulus-dependent manner.[17] In a recent study, Yuan et al.[15] showed that high CO levels during normoxia inhibit H2S production through protein kinase G (PKG)-dependent phosphorylation of Ser377 of CSE. Reduced CO generation during hypoxia relieves the inhibition of CSE, leading to increased H2S generation in the CB.[15] CSE inhibitors and CSE knockout mice exhibit impaired glomus cell and sensory nerve and breathing response to hypoxia.[17] These findings suggest that low sensory discharge during normoxia is due to inhibition of H2S production by high levels of CO generated by HO-2 and that the increased sensory nerve activity by hypoxia is due to relieving inhibition of H2S synthesis by CO [Figure 1].
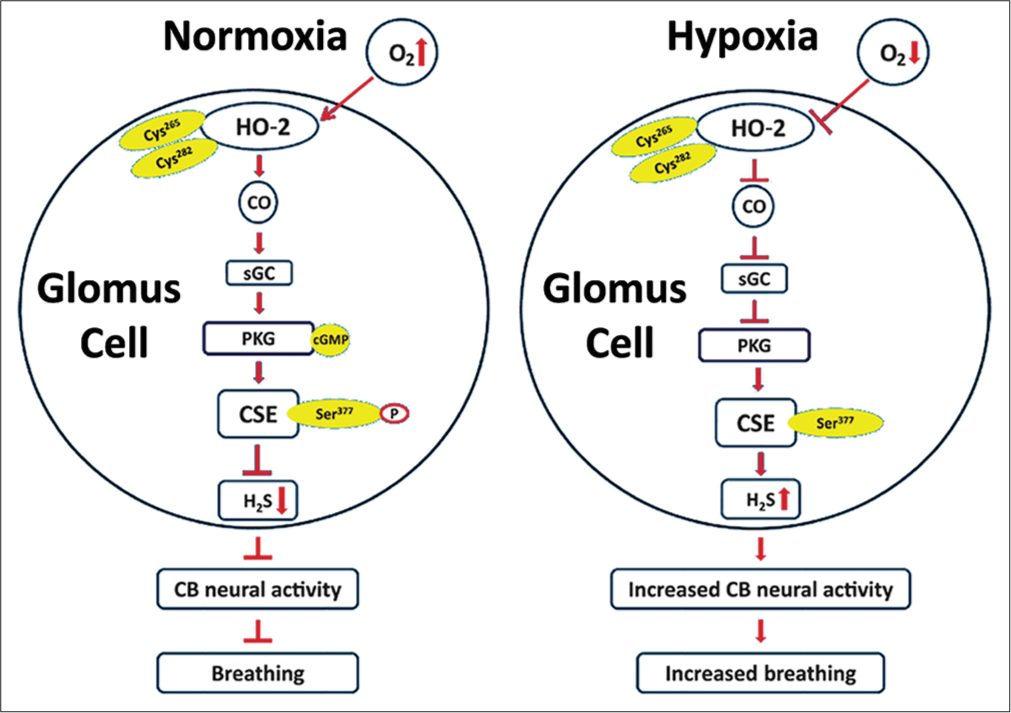
- Schematic presentation of the signalling pathways associated with innate O2 sensing by the carotid body (CB) glomus cells involving interplay between three gases—O2, CO and H2S and their impact on CB neural activity and breathing. Cys265 and Cys282 are located in the heme regulatory motif of heme oxygenase (HO)-2. Ser377 is the target residue in the putative PKG recognition sequence in cystathionine gamma-lyase (CSE) (Adapted from Yuan et al., Science Signalling, 2015, 8:373).
TRANSLATIONAL SIGNIFICANCE OF INNATE O2 SENSING BY THE CB
CB sensory nerve activity is transmitted to brainstem neurons producing reflex stimulation of breathing and sympathetic nerve activity. Heightened CB chemo reflex has been implicated in several diseases associated with autonomic dysfunction.
Obstructive sleep apnoea (OSA)
OSA is a widespread respiratory disorder affecting 20–30% of men and 10–15% of women.[19,20] Intermittent hypoxia (IH) is a hallmark manifestation of OSA. HO-2 knockout mice exhibit OSA, which could be prevented with either genetic or pharmacological blockade of H2S synthesis by CSE.[21,22] IH-treated rodents exhibit enhanced CB sensitivity to hypoxia, elevated H2S and reduced CO levels in the glomus tissue.[22] IH-evoked CB hypersensitivity to hypoxia, increased sympathetic nerve activity and hypertension[22] are prevented with pharmacological blockade of H2S synthesis.[22]
Neurogenic hypertension
CB chemo reflex is augmented in humans with essential hypertension.[23] Spontaneous hypertensive rats, a rodent model of neurogenic hypertension, manifest CB hypersensitivity to hypoxia and elevated H2S levels in the CB.[16] Pharmacological blockade of H2S synthesis lowers BP in SH rats[16] indicating pharmacological blockade of H2S synthesis might be of therapeutic value in normalising BP in neurogenic hypertension.
Congestive cardiac failure (CCF)
Patients with CCF exhibit elevated sympathetic nerve activity, which contributes to progression of CCF and mortality.[24,25] Enhanced CB chemo reflex is an important contributor to increased sympathetic nerve activity in experimental models of CCF.[26] H2S synthesis inhibitor normalises CB hypersensitivity to hypoxia and prevents the increased sympathetic nerve activity in experimental models of CCF.[26]
ADAPTIVE O2 SENSING
Whereas certain cells such as glomus cells of the CB exhibit ‘innate’ O2 sensing, every mammalian cell responds to chronic hypoxia that is, ‘adaptive’ O2 sensing. As early as 1890, it was recognised hypoxia associated with high altitude increases red cell production, facilitating the O2-carrying capacity of blood.[27] Subsequent studies showed hypoxia increases erythropoietin, which stimulates RBC production. In early 1990s, Dr. Gregg L. Semenza at the Johns Hopkins University School of Medicine was investigating the molecular basis of oxygen-regulated human erythropoietin (EPO) gene expression. He identified a nuclear factor, known as hypoxia-inducible factor 1 (HIF-1), which binds to hypoxic response element (HRE) sequence in DNA initiating transcriptional activation of the EPO gene.[28] HIF-1 complex is a heterodimer composed of an O2-regulated HIF-1α subunit[29,30] and constitutively expressed HIF-1β subunit. In presence of O2, HIF-1α is rapidly degraded and accumulated in cells only under hypoxia. How does O2 regulate HIF-1α protein? Studies by Drs. Kaelin at Harvard and Sir Peter Ratcliffe at Oxford showed von-Hippel-Lindau (VHL) physically interacts with HIF-1α protein, leading to its degradation in normoxia.[31,32] How do O2 levels regulate VHL and HIF-1α protein interaction? Ratcliffe’s group showed that prolyl hydroxylase domain proteins (PHD 1, 2 and 3) catalyse the hydroxylation of proline residues in HIF-1α.[33] PHDs are highly sensitive to O2 with an apparent Km of ~170 mmHg. Low affinity for O2 suggests that even a small reduction in O2 can inhibit PHDs, leading to reduced prolyl hydroxylation and less binding to VHL, thereby resulting accumulation of HIF-1α protein. The increased HIF-1α protein diamerzes with HIF-1β and the HIF-1 complex enters the nucleus and binds to HREs on DNA to drive gene transcription [Figure 2]. Following the initial discovery of HIF-1, two related proteins were identified including HIF-2α (also known as endothelial PAS domain protein [Epas]-1) and HIF-3α. HIF-1 is expressed in all mammalian tissues and cell types, whereas HIF-2α expression is restricted to specific cell types, including developing blood vessels and lungs. VHL and PHD signalling is utilised for O2-dependent regulation of both HIF-1α and HIF-2α and these signalling pathways are evolutionarily conserved. The fundamental discoveries by Drs. Semenza, Ratcliffe and Kaelin established the molecular basis of ‘adaptive’ O2 sensing and were recognised with Nobel Prize in Physiology and Medicine in 2019.

- Hypoxia-inducible (HIF) signalling in adaptive O2 sensing. Oxygen-dependent regulation of HIF-1α. In normoxic conditions, prolyl hydroxylase proteins (PHD) hydroxylate prolines (OH) residues in the oxygen-dependent degradation domain of the HIF-1α protein. The hydroxylated prolines are recognised by the von Hippel-Lindau protein (VHL) and ubiquitinated (Ub) for degradation by the proteasome. In hypoxic conditions, PHDs are inhibited resulting in accumulation of HIF-1α protein and HIF-1β is dimerised and translocated to the nucleus, binds to the hypoxia-responsive element (HRE) in DNA and activate transcription of genes regulated by hypoxia (Adapted from Cerychova and Gabriela, Frontiers in Endocrinology, 2018, 9:460).
HIF-1 mediates transcriptional regulation of hundreds of genes in response to hypoxia, including genes encoding proteins that control angiogenesis (i.e., O2 delivery) as well as genes encoding enzymes and transporters that control energy metabolism (i.e., O2 utilisation).
SIGNIFICANCE OF ADAPTIVE O2 SENSING
Hypoxia associated with high altitude sojourn leads to a series of adaptations, including ventilatory acclimatisation to hypoxia (VAH), characterised by a progressive increase in baseline ventilation. Failure to hyperventilate at high altitudes leads to severe hypoxemia and pulmonary and brain oedema. VAH is severely impaired in mice partially deficient in HIF-1α.[34] Although Tibetan and Andean populations reside at high altitudes for thousands of years, they adapted differently to hypoxia. Tibetans developed increased ventilatory capacity, whereas Andeans have higher haemoglobin concentrations than lowlanders.[35] Absence of polycythemia in Tibetans suggests that they adapted more effectively to hypoxia. Independent studies from different research groups reported a remarkable correlation between genetic variation of HIF signalling pathways and physiological adaptation in Tibetan and Andean populations. Non-elevated haemoglobin phenotype in Tibetans was associated with selected haplotypes at the EGLN1 and PPARA loci, which are HIF-1 target genes that encode prolyl hydroxylase PHD2 and peroxisome proliferator-associated receptor, respectively.[36] Genome-wide analysis of Tibetans revealed a high correlation between the presence of single nucleotide polymorphisms at the EPAS1 locus (which encodes HIF-2α) and non-elevated haemoglobin concentrations in this population.[37,38] These studies provide compelling evidence that HIF signalling plays a major role in evolutionary and physiological adaptations to chronic hypoxia. Perhaps, the most important significance of HIFs lies in cancer biology. Tumours are hypoxic and exhibit elevated HIF proteins. HIF activation promotes angiogenesis in tumours facilitating metastasis. Therefore, blockade of HIF signalling inhibiting angiogenesis has the potential to block metastasis. Indeed, recent studies have shown that targeted pharmacological blockade of HIF-2α reduces tumour size in certain kidney cancers[39] and these drugs are in clinical trials. Thus, the adaptive O2 sensing associated with discovery of HIF signalling has enormous physiological and pathophysiological significance.
References
- Ueber die Ursache der athembewegungen, sowie der dyspnoë und apnoë. Pflügers Arch Gesamte Physiol Meschen Tiere. 1868;1:61-106.
- [CrossRef] [Google Scholar]
- Sur la structure et l'innervation de la glande intercarotidienne (glomus caroticum) de l'homme et des mammiferes et sur un nouveau systeme de l'innervation autonome du nerf glossopharyngien. Trav Lab Rech Biol. 1926;24:365-432.
- [Google Scholar]
- Technique pour la coloration du système nerveux quand il est pourvu de ses étuis osseux. Trav Lab Rech Biol. 1925;23:427-46.
- [Google Scholar]
- Evolución de los ganglios simpáticos vertebrales y prevertebrales. Conexiones y citotectonia de algunos grupos de ganglios en el niño y hombre adulto. Trab Lab Invest Biol Univ Madrid. 1923;20:113-208.
- [Google Scholar]
- Sinus caroticus and respiratory reflexes: I. Cerebral blood flow and respiration. Adrenaline apnoea. J Physiol. 1930;69:254-66.
- [CrossRef] [PubMed] [Google Scholar]
- Le sinus carotidien, zone reflexogene regulatrice du tonus vagal cardiaque du tonus neurovasculaire et deI'adrenalinosecretion. Arch Int Pharmacodyn Ther. 1929;35:269-313.
- [Google Scholar]
- Sur les modifications directes et sur la regulationreflexede l'activitie du centre respiratory de la tete isolee du chien. Arch Int Pharmacodyn Ther. 1927;33:273-372.
- [Google Scholar]
- Recherches physiologiques et pharmacologiques sur la tête isolée et le centre vague du chien. I: Anémie, asphyxie, hypertension, adrénaline, tonus pneumogastrique. Arch Int Pharmacodyn. 1925;30:145.
- [Google Scholar]
- Towards the sensory nature of the carotid body: Hering, de castro and heymansdagger. Front Neuroanat. 2009;3:23.
- [CrossRef] [PubMed] [Google Scholar]
- Peripheral chemoreceptors: Function and plasticity of the carotid body. Compr Physiol. 2012;2:1, 41-219
- [CrossRef] [Google Scholar]
- Role of alpha 2-adrenergic receptors in the carotid body response to isocapnic hypoxia. Respir Physiol. 1991;83:353-64.
- [CrossRef] [PubMed] [Google Scholar]
- Depression of hypoxic hyperventilation in man by sudden inspiration of carbon monoxide In: Torrance RW, ed. Arterial Chemoreceptors. Blackwell: Oxford; 1968. p. :145-50.
- [Google Scholar]
- Carbon monoxide: A role in carotid body chemoreception. Proc Natl Acad Sci U S A. 1995;92:1994-7.
- [CrossRef] [PubMed] [Google Scholar]
- Protein kinase G-regulated production of H2S governs oxygen sensing. Sci Signal. 2015;8:ra37.
- [CrossRef] [PubMed] [Google Scholar]
- Inherent variations in CO-H2S-mediated carotid body O2 sensing mediate hypertension and pulmonary edema. Proc Natl Acad Sci U S A. 2014;111:1174-9.
- [CrossRef] [PubMed] [Google Scholar]
- H2S mediates O2 sensing in the carotid body. Proc Natl Acad Sci U S A. 2010;107:10719-24.
- [CrossRef] [PubMed] [Google Scholar]
- Hydrogen sulfide activates the carotid body chemoreceptors in cat, rabbit and rat ex vivo preparations. Respir Physiol Neurobiol. 2015;208:15-20.
- [CrossRef] [PubMed] [Google Scholar]
- Increased prevalence of sleep-disordered breathing in adults. Am J Epidemiol. 2013;177:1006-14.
- [CrossRef] [PubMed] [Google Scholar]
- Hypoxia-inducible factors and obstructive sleep apnea. J Clin Invest. 2020;130:5042-51.
- [CrossRef] [PubMed] [Google Scholar]
- Complementary roles of gasotransmitters CO and H2S in sleep apnea. Proc Natl Acad Sci U S A. 2017;114:1413-8.
- [CrossRef] [PubMed] [Google Scholar]
- The sympathetic nervous system and blood pressure in humans: Implications for hypertension. J Hum Hypertens. 2012;26:463-75.
- [CrossRef] [PubMed] [Google Scholar]
- Role of the carotid body in the pathophysiology of heart failure. Curr Hypertens Rep. 2013;15:356-62.
- [CrossRef] [PubMed] [Google Scholar]
- Carotid body function in heart failure. Respir Physiol Neurobiol. 2007;157:171-85.
- [CrossRef] [PubMed] [Google Scholar]
- Inhibition of hydrogen sulfide restores normal breathing stability and improves autonomic control during experimental heart failure. J Appl Physiol. 2013;114(1985):1141-50.
- [CrossRef] [PubMed] [Google Scholar]
- Ueber das gesetz der dissociation des oxyhämoglobins und über einige daran sich knüpfenden wichtigen fragen aus der biologie. Arch Anat Physiol. 1890;2:1-27.
- [Google Scholar]
- A nuclear factor induced by hypoxia via de novo protein synthesis binds to the human erythropoietin gene enhancer at a site required for transcriptional activation. Mol Cell Biol. 1992;12:5447-54.
- [CrossRef] [PubMed] [Google Scholar]
- Hypoxia-inducible factor 1 is a basic-helix-loop-helix-PAS heterodimer regulated by cellular O2 tension. Proc Natl Acad Sci U S A. 1995;92:5510-4.
- [CrossRef] [PubMed] [Google Scholar]
- Purification and characterization of hypoxia-inducible factor 1. J Biol Chem. 1995;270:1230-7.
- [CrossRef] [PubMed] [Google Scholar]
- The tumour suppressor protein VHL targets hypoxia-inducible factors for oxygen-dependent proteolysis. Nature. 1999;399:271-5.
- [CrossRef] [PubMed] [Google Scholar]
- Von Hippel-Lindau disease: Insights into oxygen sensing, protein degradation, and cancer. J Clin Invest. 2022;132:e162480.
- [CrossRef] [PubMed] [Google Scholar]
- C. Elegans EGL-9 and mammalian homologs define a family of dioxygenases that regulate HIF by prolyl hydroxylation. Cell. 2001;107:43-54.
- [CrossRef] [PubMed] [Google Scholar]
- Defective carotid body function and impaired ventilatory responses to chronic hypoxia in mice partially deficient for hypoxia-inducible factor 1 alpha. Proc Natl Acad Sci U S A. 2002;99(2):821-6.
- [CrossRef] [PubMed] [Google Scholar]
- Two routes to functional adaptation: Tibetan and Andean high-altitude natives. Proc Natl Acad Sci U S A. 2007;104(Suppl 1):8655-60.
- [CrossRef] [PubMed] [Google Scholar]
- Identifying positive selection candidate loci for high-altitude adaptation in Andean populations. Hum Genomics. 2009;4:79-90.
- [CrossRef] [PubMed] [Google Scholar]
- Gain-of-function EGLN1 prolyl hydroxylase (PHD2 D4E: C127S) in combination with EPAS1 (HIF-2α) polymorphism lowers hemoglobin concentration in Tibetan highlanders. J Mol Med (Berl). 2017;95:665-70.
- [CrossRef] [PubMed] [Google Scholar]
- Natural selection on EPAS1 (HIF2alpha) associated with low hemoglobin concentration in Tibetan highlanders. Proc Natl Acad Sci U S A. 2010;107:11459-64.
- [CrossRef] [PubMed] [Google Scholar]
- Phase I dose-escalation trial of PT2385, a first-in-class hypoxia-inducible factor-2α antagonist in patients with previously treated advanced clear cell renal cell carcinoma. J Clin Oncol. 2018;36:867-74.
- [CrossRef] [PubMed] [Google Scholar]